Turbulence is both a fundamental research problem and one of the most difficult engineering challenges of high importance to the nuclear deterrence program because it affects the vibrational loading and heating of reentry vehicles (RVs). The primary source of vibrational loading and heating during reentry is the turbulent boundary layer created by gas flowing over the vehicle’s surface. Thermal-protection-system (TPS) materials that are used to coat RVs are often fibrous and may ablate due to extreme heating rates, creating a rough and permeable surface. This surface has the potential to significantly alter the turbulent boundary layer and thus alter the heating rate and vibrational loading. This in turn could affect vehicle performance. Consequently, there is great interest in the ability to accurately predict high-speed turbulent flows over RVs. The ability to make such predictions, however, is limited by the extreme complexity posed by the multi-scale nature of turbulence.
Simulating turbulence at the molecular level
Numerical simulations play a vital role in quantitatively understanding and predicting the effects of high-speed turbulent flows because of the difficulty associated with performing experimental measurements. Turbulent flows are almost universally simulated using the continuum Navier-Stokes (NS) equations, and their ability to accurately describe all length and time scales larger than molecular scales is rarely questioned. Typical NS simulations, however, do not include highly non-equilibrium effects or simulate molecular-level processes and neglect their impact on high-speed turbulence.
In contrast, the direct simulation Monte Carlo (DSMC) method provides a high-fidelity representation of non-equilibrium gas behavior by directly simulating the motion of computational molecules. A computational “molecule” in DSMC may represent thousands of real molecules, leading to a more tractable simulation. DSMC has been recently shown to provide a computationally viable and physically superior way to investigate the relationship between molecular-scale processes and hydrodynamic-scale turbulence in a gas. Molecular methods, including DSMC, are computationally intense. However, petascale computational resources enable DSMC to accurately simulate non-equilibrium transitional and turbulent flows that contain length and time scales ranging from microscopic (molecular) to macroscopic (hydrodynamic).
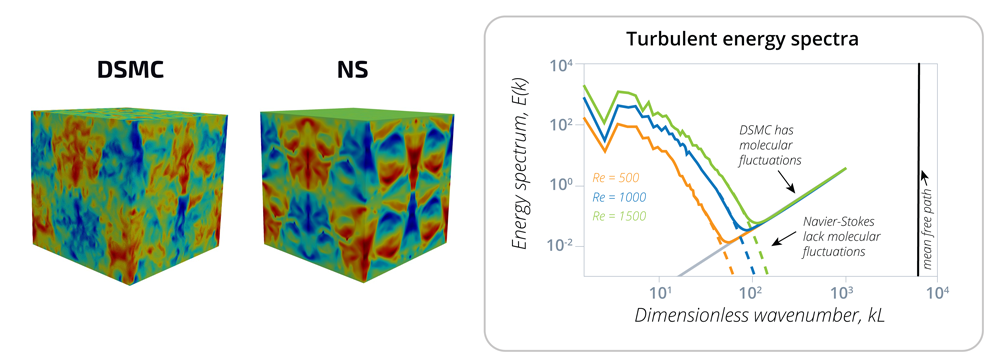
For the last decade, Sandia has been developing SPARTA, a state-of-the-art massively parallel DSMC code. SPARTA is the world’s premier open-source DSMC code, with hundreds of users worldwide. It is used extensively by academia, industry and space agencies. SPARTA has been developed to ensure portable performance and efficient use of Central Processing Unit (CPU) and Graphics Processing Unit (GPU) machines, enabling extreme-scale science on current and future supercomputer platforms. Turbulence simulations in this work typically utilized one third of National Nuclear Security Administration’s (NNSA’s) Advanced Technology System (ATS)-2 Sierra supercomputer at Lawrence Livermore National Laboratory, and several simulations were also performed using the entirety of the ATS-1 Trinity supercomputer at Los Alamos National Laboratory. Turbulence simulations currently run on ATS-3 Crossroads. SPARTA is also being ported to ATS-4 El Capitan and is being used as a benchmark for the future NNSA ATS-5 supercomputer because of its exceptional parallel performance and large usage on current ATS supercomputers.
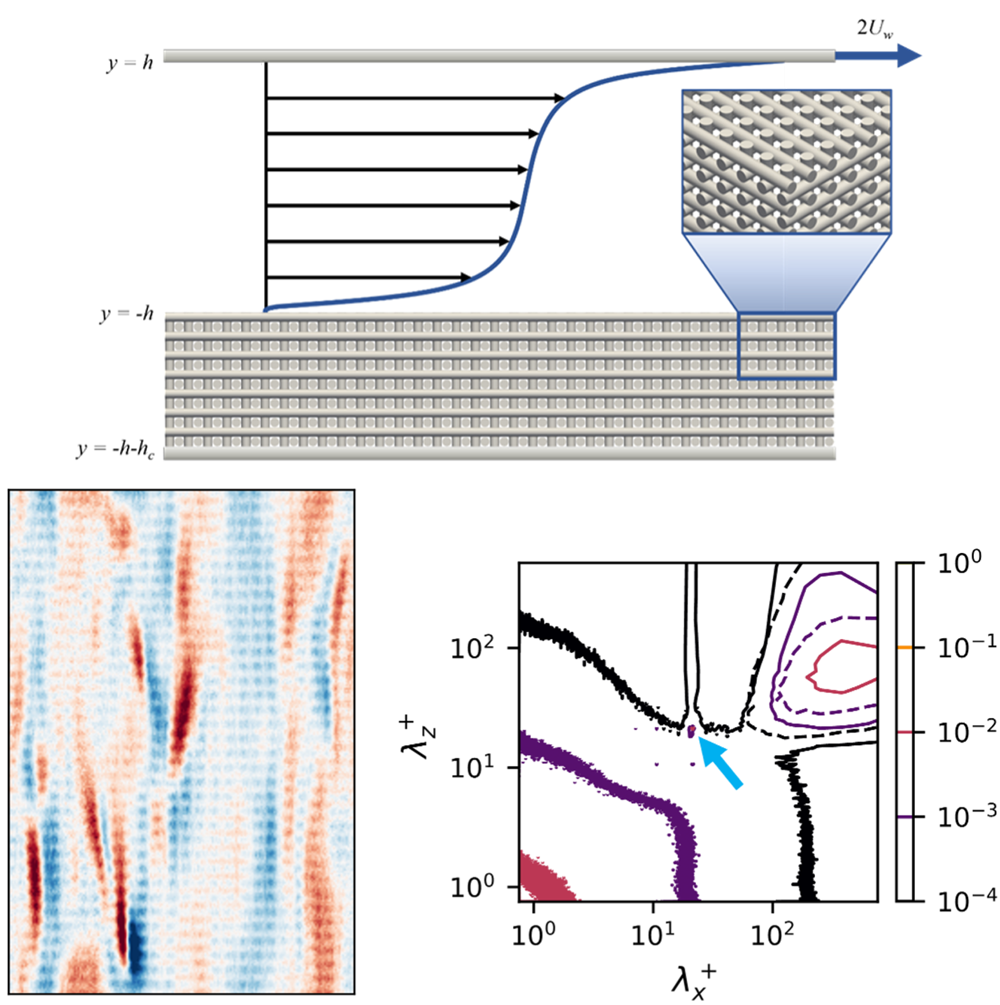
An important question that DSMC is well-suited to tackle is: What length scales in turbulent flows are affected by molecular-level phenomena? To address this question, decaying turbulence was simulated using SPARTA, and the results were compared with NS simulations (see Figure 1). The simulations revealed that the NS equations do not accurately represent scales in the so-called dissipation range in turbulent gas flows because they neglect molecular fluctuations. It was found that the DSMC turbulent kinetic energy spectra grow quadratically with wavenumber in the dissipation range due to molecular fluctuations (see Figure 1, bottom), which is in agreement with theoretical predictions, whereas the NS spectra decay exponentially. The length scale for which molecular fluctuations begin to dominate is notably much larger than the gas-molecular mean free path, specifically, in a regime that the NS equations are widely believed to describe.
Turbulent boundary layers over rough and permeable surfaces, like those found on RVs covered in TPS materials, are another example of turbulent flow where molecular-level phenomena play a significant role. The length scales associated with the roughness and permeability of typical TPS materials can notably be comparable to the gas-molecular mean free path, which itself may not be small compared to other characteristic flow length scales.
Recent DSMC simulations of turbulent flow over an idealized permeable fibrous substrate representative of TPS materials (see Figure 2, top) demonstrated that the near-wall turbulence is modulated by the permeable substrate with a wavelength equal to the pore spacing (see Figure 2, bottom). The flow within the substrate also shows significant rarefaction effects, resulting in an effective permeability that is significantly larger than the intrinsic permeability. This higher effective permeability means that gas can move more easily through the surface than the NS equations predict, which could have significant implications for thermal transport and surface chemistry in TPS materials.
Future work on high-speed turbulent flows
These investigations demonstrate the ability of DSMC, in particular of SPARTA, to simulate turbulent flows at the molecular level and highlight previously unappreciated limitations of the NS equations. This work opens a new path of research where molecular-level simulations can be used to quantitatively understand phenomena in high-speed turbulent flows that are currently inaccessible to more conventional methods. Because of SPARTA’s excellent parallel performance, the advent of exascale supercomputers such as Oak Ridge National Laboratory Frontier, Argonne National Laboratory Aurora, and the future NNSA El Capitan will allow DSMC to push the state of the art in turbulence research even further.