Experiments at Sandia’s Z Machine—the largest pulsed-power device in the world today—generate some of the most extreme laboratory conditions on our planet, providing glimpses into the behavior of planet cores, asteroid impacts and accretion around black holes; however, the enormous pressure, temperature and radiation intensity necessary to study these phenomena are often destructive to diagnostics and other equipment. The success of future fusion energy facilities will rely on wall materials and devices designed to operate within such harsh conditions. Potential insults include radiation, shock, heating, debris, magnetic fields and even vibration akin to small earthquakes—necessitating computer models of experimental designs that incorporate many different types of physical effects.
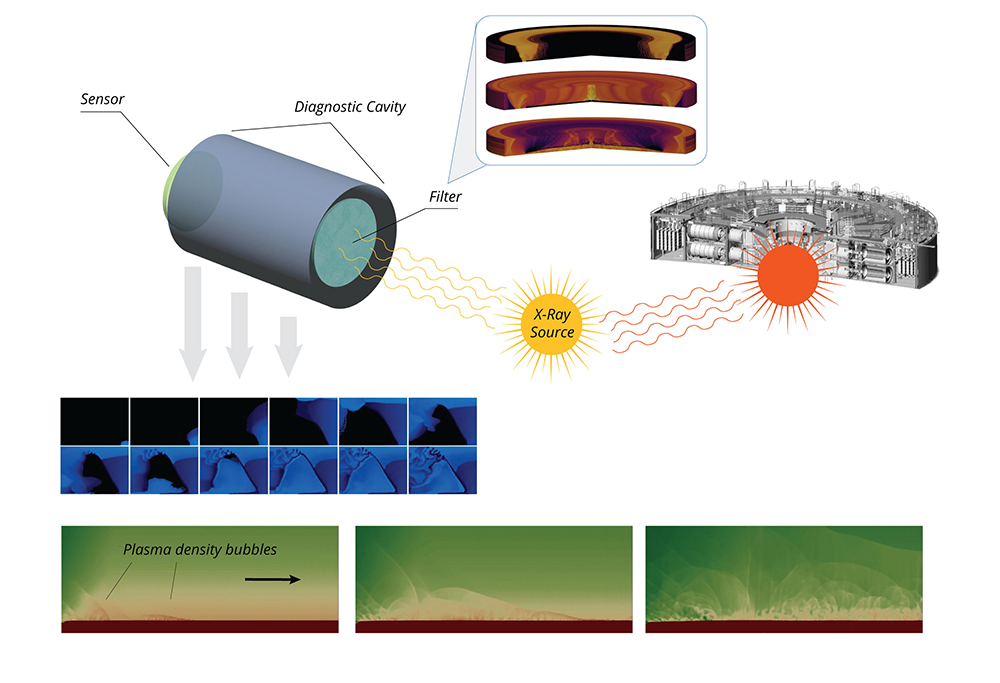
Recent advances at Sandia have enabled accurate, high-fidelity, large-scale simulations of many of these processes to be conducted for the first time. Central to these developments is ALEGRA (Arbitrary Lagrangian Eulerian General Research Application), a code with new algorithms that expand Sandia’s capabilities. Unlike other codes customized for one application, ALEGRA was envisioned to allow simultaneous simulation of many different, interwoven processes. For example, ALEGRA can simulate the motion of individual x-ray photons as they scatter through a material, leaving tracks of energy that alter its flow—a process called radiation-hydrodynamics. As the flow is altered, the location and magnitude of the energy deposition is also altered. These processes can occur quickly. The cumulative effect of many photons striking a surface can induce temperatures up to 100,000K, ejecting plumes of hot gas at hypersonic speeds and altering the flow within nanoseconds.
A key advance is the ability to perform stochastic radiation-hydrodynamic calculations on axisymmetric geometries to allow rapid assessment of experimental cavities. Figure 1 shows an external filter that protects and controls illumination of an internal experiment. Radiation from the center of the Z Machine heats the x-ray filter enough to form an ionized plasma, which changes its ability to transmit x-rays. Some of the x-rays scatter into the nearby cavity walls where they generate a highly energetic plasma that travels at speeds up to 80 km/s.
This plasma overtakes the filter, leading to its complete destruction within a few millionths of a second (see Figure 2). Understanding the dynamics of these events is crucial for ensuring that the filter will maintain the desired illumination and protect the experiment inside the cavity.
Figure 2. Fully coupled radiation-hydrodynamics simulation using ALEGRA to predict the time of destruction of an x-ray filter used in an experimental cavity at the Z Machine.
The new capabilities have also been used to predict complex plasma flows inside experiments. As shown in Figure 3, hot plasma from an irradiated corner of the material begins to fill a void in the nearby vacuum. At first, the plasma is unimpeded and expands quickly, rebounding off the fixture walls. But the corner, which was superheated by the radiation, continues to eject a plume of hypersonic gas. The collision of plasma fronts restricts the expansion to a triangular region, while also inducing the fanciful vorticity seen around the edges. These plasma dynamics, which can only be understood through multiphysics simulation, influence the strength of optical diagnostic beams that must transmit through the cavity. ALEGRA simulations have enabled the design of such novel experiments.
Figure 3. Plasma evolution within an experimental cavity.
Radiation-hydrodynamic simulations have also been used to improve our understanding of surface erosion from intense radiation bursts, such as those that would be needed to deflect an asteroid on a collision-course with Earth, or to predict the reliability of a containment wall in a fusion reactor. Certain Z Machine experiments can be used to emulate these processes. Figure 4 shows a simulation of an experiment heating the edge of a metallic disc with an intense x-ray pulse. Although the entire surface vaporizes, perturbations near the outer edge cause a series of plasma density bubbles to race toward the center of the cylindrical panel. These density fluctuations cause different rates of erosion to occur across the disc. Understanding the interactions of these plasma jets with material surfaces may be particularly important for deflecting asteroids, many of which have rough surfaces and uneven topology.
Figure 4. Plasma density bubbles following x-ray irradiation of a metal surface. Disturbances in plasma density race toward the center of a cylindrical disc, leading to differences in surface erosion rates.
Sandia’s broad mission space requires flexible, multiphyics codes that can tackle a wide range of problems. Toward that end, radiation-hydrodynamic capabilities have been further extended by coupling ALEGRA to SCEPTRE under the RAMSES code suite. This coupling was the result of a multi-year collaboration between the code teams, combining over two decades of research and development. Like ALEGRA, SCEPTRE tracks the movement of photons in a material, but it also calculates the trajectories of electrons jetisoned from atoms that are struck by x-rays. Other collisions will simply change the direction of a photon, but some of the energy is lost. SCEPTRE contains advanced algorithms that recalculate the resulting change in the energy spectrum of the x-ray field as it propagates through a material. The result is increased accuracy in calculating where energy is deposited, which affects erosion of walls and coatings. SCEPTRE can also simulate intense streams of electrons impinging on materials during particle accelerator experiments.
Results from SCEPTRE are comm-unicated back to ALEGRA in real time using a multiple program, multiple data (MPMD) algorithm, which works like an exchange broker: each code contributes new information about the material state, which is updated in real-time as the material evolves. Besides increasing accuracy, this strategy allows developers to work on each code independently, streamlining future improvements. A recent upgrade to the x-ray absorption library in ALEGRA allows calculations to be performed for mixtures of any elements in the periodic table, enabling agile design of materials and experiments. Another critical advance is the ability to apply SCEPTRE to selected regions of a material, which has led to a 16x reduction in computational cost and enabled larger scale, multiphysics solutions.
Future developments will further improve the capability, usability and flexibility of ALEGRA. Work is underway to more seamlessly couple ALEGRA to codes that use different numerical schemes to represent the same geometry, and to allow more seamless transfer of data between different models. These advances will expand the number of ways that models for different physical processes can be combined to solve complex, multiphysics problems.